Part 3: What is the yield of genetic transformation?
What does this all mean for a therapeutic cell sorter? Future cell therapies may be 10-fold more demanding in terms of sort yield and purity than current therapies, thus reinforcing the need for improved therapeutic cell sorting.
What is the yield of genetic transformation?
This is the third post in this series looking at the question of how many cells will future therapeutic cell sorters need to be able to sort. The two previous posts looked at how many cells are needed for a T cell therapy, and how many fold such cells can be expanded in vitro.
But the most exciting aspect of T cell therapy currently is not cell dose, nor cell expansion, but the genetic modification of cells. This has resulted in dramatic advances in the cell therapy field in recent years. The efficiency of genetic modification also crucially affects how many cells will need to be sorted in a therapeutic setting.
Transduction technology, based on viral vectors, is now fairly established for the leading CAR T cell therapies, and the major labs have published the efficiencies they have achieved. However, the results also show that transduction efficiency varies hugely between patients.
Scientists are also experimenting with new genetic modification techniques, such as transposons like “Sleeping Beauty” or new gene editing technologies like ZFNs, TALENs and CRISPR-Cas9, which offer important advantages over viral transduction.
As the cell and gene therapy field advances, the transfection efficiencies of future therapies are unknown, but it is likely that new CAR concepts will throw up new challenges for transfection.
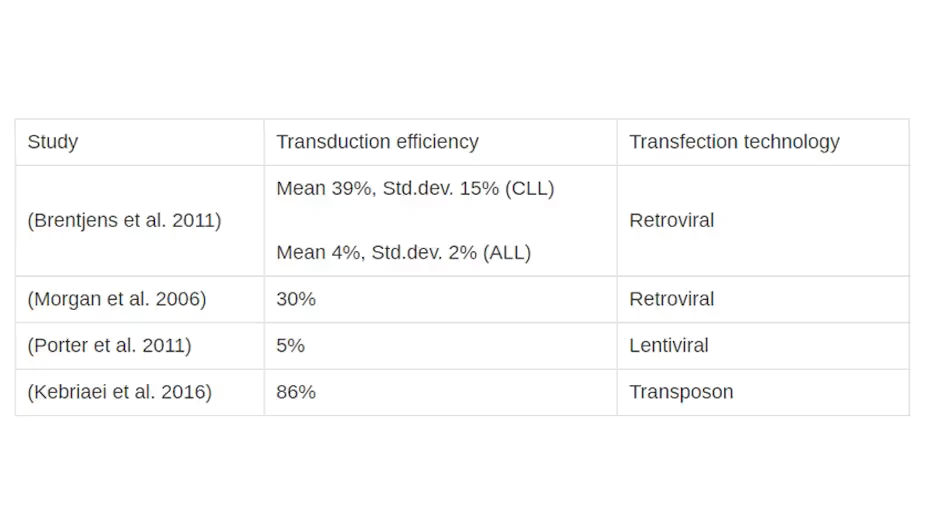
The research literature provides insights into gene transfer technologies and efficiencies in clinical trials. Davila et al. (2014) have produced an excellent summary of the gene transfer technologies used in relevant clinical trials up to 2014. Table 1 shows a selection of the average transduction efficiencies achieved in key studies by retroviral / lentiviral transfer. Brentjens et al. (2011) provide even more data, citing transduction efficiencies for every patient in the trial.
Clearly, the results are hugely variable: efficiencies ranging from around 4% to 70% have been reported.
Viral-mediated transfer (by retroviruses or lentiviruses) has become the method of choice to achieve more stable and efficient gene transfer than physical methods, such as electroporation and lipofection, would allow. While these viruses are also feared as potential causes of cancer, and must be subject to stringent manufacturing controls to avoid retrovirus transmission, the safety data from many years of cell therapy trials is encouraging (Scholler et al. 2012).
However, ingenious research has continued to deliver further cell modification methods. Kebriaei et al. (2016) have demonstrated a very high CAR transfection efficiency of 86% with the Sleeping Beauty transposon system. This may improve the safety of CAR T-cell therapy and allow greater control in the transformation of cells.
In the meantime, researchers have started using gene editing techniques such as ZFNs, TALENs and CRISPR-Cas9 which offer the ability to knock out specific genes and insert genes at defined locations in the cell’s DNA (Fesnak, June, and Levine 2016; Chen 2015). Gene knock-out efficiencies of 40% and knock-in efficiencies of 20% have been demonstrated in T-cells with the Cas9 system (Schumann et al. 2015). Although gene editing techniques currently give significantly lower yields than viral transduction or transposons, they are the only way to knock out specific genes or control the location of gene insertion.
The demands on gene transfer technology are also increasing with novel and more sophisticated CAR concepts. Fesnak, June, and Levine (2016) categorised ten new CAR approaches, as set out in their Figure 5 below. All these innovative concepts potentially reduce expression efficiency and would thus require better therapeutic cell sorting.
- Some approaches require co-expression (or tandem expression) of two proteins: (a) TRUCK, (c) self-driving CAR, (g) marked CAR, (j) safety CAR. The efficiency of current gene transfer technology is lower for such constructs.
- Some approaches are based on more complicated single CARs: (f) conditional CAR, (h) tanCAR (i) dual CAR. These potentially lower the transduction/expression efficiency of existing techniques.
- Some approaches require specific knock-out of native proteins: (b) universal (allogenic) CAR, and (d) armoured CAR. These require gene editing in addition to CAR expression, so they have a low total efficiency using current techniques.
- The remaining approach, (e) self-destruct CAR, is based on transfection methods that afford lower stability and efficiency than viral delivery.
Despite these newer CAR approaches presenting a greater challenge in terms of transformation efficiency, many of them are also more likely to require well-defined cell populations or even near-100% purity of the modified cells. An example for the latter requirement is allogenic T-cell therapy, where nearly 100% of the donor’s native T-cell receptors must be eliminated to avoid graft versus host disease (GvHD).
The recent high-profile application of TALEN-engineered universal CAR19 T cells in B-cell acute lymphoblastic leukaemia (Qasim et al. 2015), used TALEN to knock out native T-cell receptors (TCR), followed by immunomagnetic TCR depletion to bring down the non-knocked-out cells to < 1% of the therapeutic dose. Moreover, future production of universal CARs may be even more demanding, both on yield, and purity, of the sorted cells.
What does this all mean for therapeutic cell sorters? While current applications already stretch the capabilities of existing cell sorters, future CAR therapies may be 10-fold more demanding in terms of sort yield and purity. This reinforces the need for improved cell sorting to support these innovative therapies.

Diagram from Fesnak, June, and Levine (2016) categorising the sophisticated CAR concepts that have emerged in recent years.
References